What's New
Using ultrasound to image bacteria in vivo: acoustic reporter genes
In vivo imaging of Bacteria with Acoustic Reporter Genes (ARGs)
Posted By Beth Kenkel
Knowing where bacteria are located within their host is often key to understanding their role in both health and disease. To observe bacteria in action, researchers have developed in vivo bacterial reporters that use fluorophores and luciferases to track bacteria in real time, but each of these reporters has its drawbacks. Acoustic reporter genes (ARGs) overcome these limitations by using gas vesicle reporters that are detectable by an inexpensive and widely available imaging platform: ultrasound.
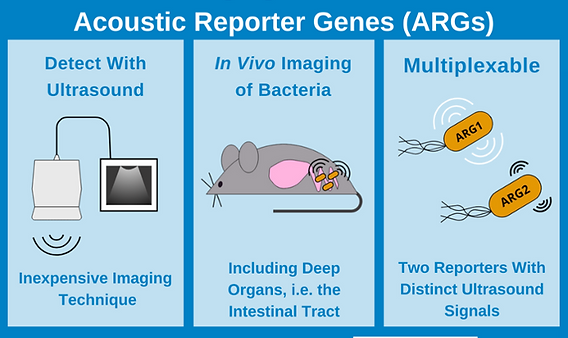
Challenges of in vivo imaging with existing bacterial reporters
-
Limited Depth of Signal/Imaging: Fluorescent reporters are not as well suited for imaging organs located deep in the body, like the gastrointestinal tract.
-
Require Administration of Substrate: Some luciferase reporters, such as Renilla luciferase (RLuc) or NanoLuc, require injection of substrate to produce bioluminescence. Substrate pharmacokinetics and biodistribution therefore introduce additional confounding factors when attempting to interpret signals from these reporters.
-
Lack anatomical context/spatial localization: In vivo imaging with fluorescent or bioluminescent reporters usually only detects the signal of the reporter and fail to pinpoint what tissue or organ this signal is coming from.
Acoustic reporter genes (ARGs)
Acoustic Reporter Genes (ARGs) are engineered gene clusters that encode proteins that form bacterial gas vesicles. These ~200nm long vesicles have hollow interiors and a protein shell that excludes water but is gas permeable. These vesicles are primarily used by water-dwelling photosynthetic bacteria and archaea to regulate their buoyancy.
The Shapiro lab recently discovered that gas vesicles also scatter sound waves and therefore produce signals in ultrasound imaging. Ultrasound is an attractive imaging platform for in vivo imaging experiments because ultrasound devices are inexpensive, widely available, and have deep tissue penetration and high spatial resolution.
By piecing together genes from gas vesicle operons from two different bacteria, Bourdeau et al created two constructs optimized for expression in E. coli: acoustic gene 1 (arg1) and acoustic gene 2 (arg2). The key difference between arg1 and arg2 vesicles is the amount of soundwave pressure required to collapse them: arg2 vesicles collapse at a lower pressure than arg1 vesicles. Following collapse, vesicles are invisible to ultrasound, which allows for differentiation of arg1 and arg2 ultrasound signals. This also means that multiplexing is possible if bacteria are sequentially exposed to acoustic pulses with high enough amplitudes to first collapse arg2 and then arg1 vesicles. By comparing ultrasound images pre-collapse and post-collapse of arg2 and then arg1, each ARG’s distinct ultrasound fingerprint can be detected. Bourdeau et al calls this method acoustic collapse. Importantly, acoustic collapse doesn’t affect bacterial viability and with 20 hours of growth following acoustic collapse E. coli re-express arg1 gas vesicles and restore their ultrasound signal.
Using acoustic reporter genes for in vivo imaging
Following in vitro characterization, Bourdeau et al performed a proof-of-concept experiment to demonstrate the most exciting application for ARG’s: in vivo imaging. Specifically, arg1 expressing E. coli aided in ultrasound imaging of the mouse gastrointestinal tract. The E. coli strain Nissle 197 (ECN) was used for these experiments because it’s a probiotic strain that can colonize the mammalian intestinal tract and has been used for 100 years to clinically treat enteric infection and inflammatory bowel conditions. In these experiments, arg1 expressing ECN were injected into the mouse colon, imaged via ultrasound, and the images compared to mice injected with ECN expressing lux. arg1 and lux reporters both allow for visualization of the bacteria, but lux imaging shows only that the bacteria localize to somewhere in the mouse abdomen. arg1 signal, however, can be overlaid onto anatomical ultrasound imaging to show where the bacteria localize in relation to internal organs. The limit of detection for arg1 expressing ECN is 109 cells/ml. Bourdaeu et al also used arg1 to in vivo image tumour-homing S. typhimurium strain ELH1301.
Pressure-Sensing Fibers Change Color When Stretched
The new material could make compression bandages more effective and easier to use
By Megan Scudellari
Posted 7 Jun 2018 | 16:30 GMT
There’s something special about the sheen of rainbow colors on oil droplets spattered on wet pavement, or on the surface of soap bubbles rising in the air.
Those colorful swirls are not the result of pigment or dye, but of light reflecting off the interfaces between the layers of water, oil or soap, and air. That effect, called “optical interference,” is also what makes the feathers of peacocks and wings of butterflies so dazzling.

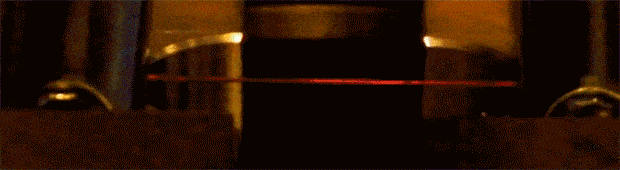
Now, a team at the Massachusetts Institute of Technology has harnessed this phenomenon to produce cool, color-changing fibers that respond to pressure. The new material, the creators say, could be used to measure force exerted on large and small areas: This could be myriad things including air pushing on an airplane’s wings or cells exerting force on their environment. But to start, the team is testing the fibers in compression bandages, the medical wrappings that help stimulate healing in certain conditions such as venous ulcers.
“I was surprised, when we talked to doctors, that there is not a [convenient tool] to tell you what the pressure is underneath these bandages,” says study author Mathias Kolle, an assistant professor in mechanical engineering at MIT. “We thought there might be a direct link between fiber color and bandage pressure if we incorporated fibers into bandages, and that worked really well.” In a small proof-of-principle study, the color of the fibers accurately predicted the amount of pressure a bandage applied to the skin beneath it.
Like other smart bandages or e-skin, the material is flexible and sensitive, yet it stands apart because it requires no electronic components or power source.
For now, the thin fibers—each about 10 times the width of a human hair—are only 6 inches long and require a fairly intensive manufacturing process. First, the researchers dissolve commonly used transparent rubbers in solvents, then pour them onto a spinning wheel. The wheel flings off most of the solution, leaving behind a thin coating. Individual coating layers are then stacked and rolled up, like a Little Debbie Swiss Roll. Light reflects off the interfaces between the layers and interacts to create colors in the visible spectrum.
With a bit of calculation, the team can change the thickness of the layers to produce whatever color tuning they desire: yellow to green, or orange to blue, for example. They can also directly correlate colors with the amount of strain, or pressure, on the fiber.